Unveiling the Sticky Secrets of Giardia: How a Parasitic Cell Masters Attachment
Posted in News Story
What’s the harm in drinking untreated water from a clean stream when you are out on a long hike? One major risk is Giardiasis, a very common parasitic disease caused when the single-cell parasite Giardia attaches to the intestinal wall and multiplies, leading to a variety of unpleasant and sometimes very dangerous symptoms. How does the cell manage to stick to the wall of the intestine, resisting the flow designed to move food through the digestive track? Fifty years ago, researchers noted that a pair of flagella are continuously beating while the cell is attached to surfaces and proposed a model where the flagella generate fluid flow between the cell and the surface, and that this flow in turn generates a pressure difference that causes the cell to stick to the substrate. Unfortunately, the researchers lacked the experimental tools to test this hypothesis. This hydrodynamic model was slowly abandoned over the subsequent years, but no serious alternative has emerged to take its place that can explain the full range of observed attachment behaviors.
An interdisciplinary team of researchers, led by Georgetown faculty members Biology Professor Heidi Elmendorf and Physics Professor Jeff Urbach used a combination of high-resolution imaging, computational modeling, theoretical analysis, innovative experimentation, to conclusively demonstrate that a version of the flow-based model accurately captures the biophysics of Giardia attachment. As described in the paper just published in PNAS Nexus, the process relies on fluid flow generated by the beating of the flagella in a tightly confined groove, acting as a microscale pump to create a low-pressure region between the cell and substrate.
“It’s something like a suction cup with a vacuum cleaner attached, […] but the vacuum cleaner is a really novel microscopic machine made up of an active biomolecular filament beating in a tightly confined space, so that fluid is continuously sucked out from under the cup” explained Urbach. The “cup” is an intricate biomolecular structure known as the Ventral Disk. The team showed that the active pumping is required to maintain low pressure under the disk because a passive suction cup can’t function in an aqueous environment on a cellular scale. Calculations included in the manuscript show that without actively removing fluid diffusion of water through the cell membrane would destroy the suction in less than a second. While Elmendorf and Urbach suspected the hydrodynamic model captured the right physics, proving it turned out to be a challenge.
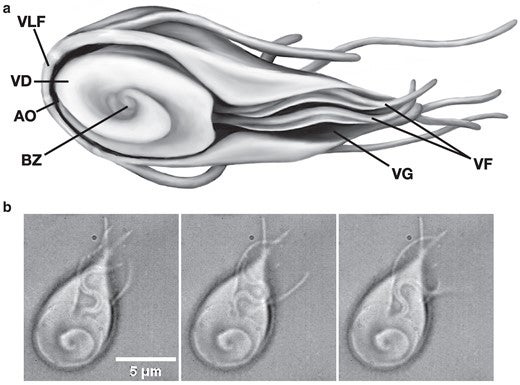
Morphology of attached Giardia. (a) Sketch of Giardia, indicating the position of the ventrolateral flange (VLF), VD, AO, BZ, VG, and ventral flagella (VF). (b) Three frames from a DIC movie (Supplementary Material, Movie S1) of Giardia attached to a glass slide, showing the VF pair beating in the space defined by the VG (60×, 50 ms between frames).
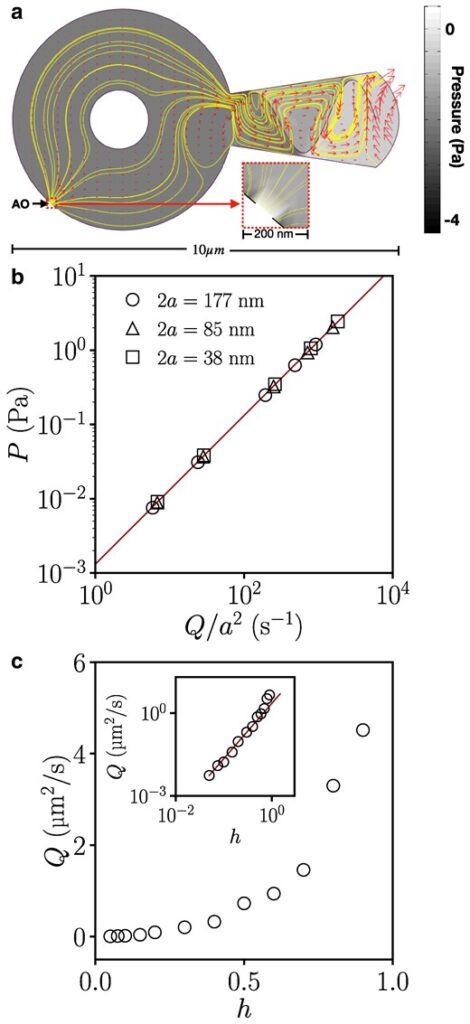
Computational model of fluid-mediated attachment. (a) Snapshot from the 2D computational model. The morphologically accurate geometry includes a circular VD with a small AO at the bottom left, corresponding to the gap in the region of overlap of the spiral array of microtubules in the VD, and a circular obstruction in the middle, representing the cell protrusion from the BZ observed in attached parasites. The ventral groove (right) contains ventral flagella beating sinusoidally, pictured for h = 0.9 (see Methods) and AO width 2a = 125 nm, showing streamlines (solid lines), velocities (vectors), and pressure relative to ambient (grayscale). Inset: Magnified view of pressure field near the AO. (b) Pressure versus flux from the computational model for different AO widths (indicated in legend) and wave amplitudes h = 0.1, 0.2, 0.5, 0.7, and 0.9. The solid line is a fit to ΔP = CηQ/a2, with C = 1.32. (c) Cycle-averaged flux versus wave amplitude. Inset: logarithmic plot of the same data, showing quadratic dependence for small h, and faster increase as h approaches unity.
When they began investigating the question of the attachment over a decade ago, the first task was to measure the effect of the Giardia on the surrounding fluid. Using advanced imaging and very small fluorescent particles, Haibei Luo (G’ 10) was able to verify that the beating flagella did in fact generate continuous flow when the cell was attached to a microscope slide in culture. Particles rarely found their way underneath the cell, but Luo and Trey Picou (G’ 17) worked with Beatriz Burrola Gabilondo (W’ 12) to use ultra-small, ultra-bright particles known as quantum dots to identify the path of fluid flow and provide the first measurements of flow speeds. The team collaborated with Engineering Professor Tom Powers from Brown University, and together they constructed both computational and analytical models to show that the measured flow was consistent with the activity of the flagella, and that the pressure changes generated by the flow are sufficient to produce the measured strength of attachment.
The last piece of the puzzle was to determine how the attachment mechanism responded to a “leaky seal” under the Giardia surface. While most lab experiments are conducted on cells attached to smooth, non-porous surfaces like glass, the wall of the intestine is covered with microvilli, creating something like a microscopic shag rug, preventing any sort of tight seal. To mimic this, the researchers replaced the hard surface with a gel where they could vary the porosity and measured how the strength of attachment changed as the substrate became more porous. Comparing their measurement with analytical calculations, they were able to confirm that the model accurately predicted just how much leakiness the pump could handle.
The researchers hope their results can guide therapeutic interventions to compromise attachment and provide a model for understanding the significance of flagellar pumping in other contexts.
“It’s very satisfying to finally have solved this puzzle, but of course it just raises more questions” said Urbach. All of their experiments and models are based on water, but the wall of the intestine is coated with mucus, a complex viscoelastic fluid that is not water like at all. The team is currently working to understand how the properties of the fluid affect the behavior of the model, and how the cells respond to the action of the pumping. More generally, the researchers expect that the insights gained from this work have potential relevance to a wide range of other biophysical systems, including other flagellated cells and other situations where filaments beat near boundaries. Finally, they hope that the results may provide inspiration for biomimetic applications for active pumping and attachment to diverse surfaces. “Nature spent millions of years refining this process,” notes Urbach “perhaps we can use it as a template for a microrobot that can find and attach to underwater surfaces and do something more useful.”
Read more HERE.